To Issue 129
Citation: Jefferies O, Margerison E, Khanolkar A, “Computational Modelling of Injection-Related Tissue Responses in Drug Delivery”. ONdrugDelivery, Issue 129 (Feb 2022), pp 18–22.
Olivia Jeffries, Elizabeth Margerison and Asmita Khanolkar introduce Oval Medical’s work on using ultrasound scanning and finite element analysis to construct a model of how tissue compresses under use of an autoinjector across patient demographics, and how this may provide insights into improved autoinjector design for more accurate injection depths, improving patient outcomes.
“Oval has developed a force-sensing ultrasound methodology for physiological analysis that enables needle lengths to be specified safely and effectively.”
INTRODUCTION
At the global level, trends continue to move medical care from hospital to the home, resulting in a growing need for at-home care for both chronic and crisis medications. This is leading to further growth areas for autoinjectors to deliver drugs and biologic products, as autoinjectors have usability advantages over other drug delivery methods, such as manual injections. Autoinjectors can enhance safety, improve dosing accuracy and improve patient compliance, especially in self-administration settings. With this significant growth, research is necessary to understand the relationship between body morphology, injection force and needle length. This can help to improve both the patient experience and the effectiveness of drug delivery devices.
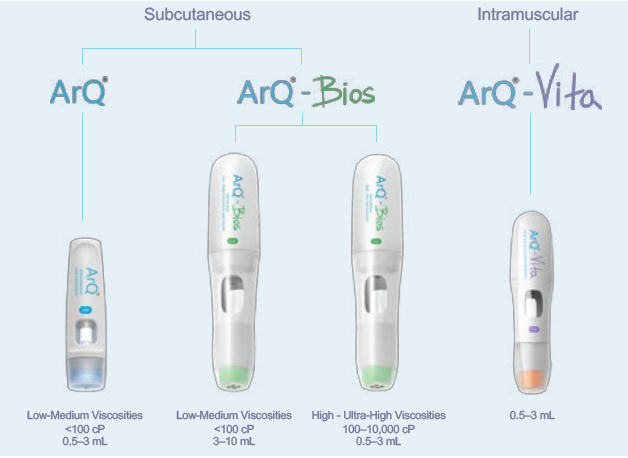
Figure 1: Subcutaneous and intramuscular delivery technologies from Oval.
Oval Medical Technologies offers a variety of autoinjector technology platforms (Figure 1), with both subcutaneous and intramuscular capabilities, to deliver a wide range of challenging novel drug formulations with high viscosities and a wide range of delivered volumes. With challenging novel formulations, complex molecules, large dosages and new delivery sites, optimising delivery along with the formulation is key for successful clinical outcomes.
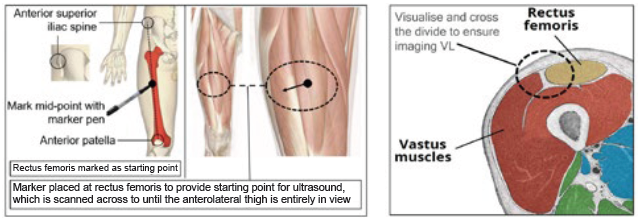
Figure 2: Identification of location for ultrasound imaging (anterolateral thigh).
A key part of successful drug administration is that the injection reaches the correct anatomical tissue. Several factors may affect the depth of injection achieved when using an autoinjector, including both injector- and patient-related factors. In addition, it is necessary to understand that the injection-related tissue responses during delivery and their correlation with patient physiology factors. Oval has developed a force-sensing ultrasound methodology for physiological analysis that enables needle lengths to be specified safely and effectively (Figure 2). This is done via characterising skin-to-muscle depth (STMD) and skin-to-bone depth (STBD) for different population groups and injection sites (Figure 3). In addition, the effects of tissue compression can be established with the use of an autoinjector.
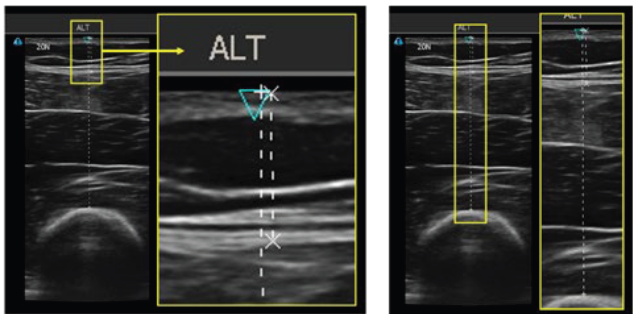
Figure 3: Ultrasound images of the thigh, indicating the skin, subcutaneous tissue, muscle and bone. Left image represents STMD and the right image is STBD.
“Consideration of the anatomical injection depth should be taken into account during the design of a drug delivery device to ensure optimal delivery of the drug, which is determined by the exposed needle length.”
Furthermore, finite element analysis (FEA) is used to model tissue compression in each of the tissue compartments at various injection sites, the results of which can be verified using Oval’s ultrasound technique. While already used extensively in other engineering fields, such as civil and aeronautical engineering, FEA is being increasingly applied within the medical field. It opens the door to many innovative and interesting methods and applications within research and design.
INJECTION SITE
The recent trend of moving treatments from hospitals to the home has been facilitated by a change from intravenous to subcutaneous and intramuscular routes of administration. This trend is driving the increasing demand for autoinjectors and, in many cases, facilitates self-administration by patients. Subcutaneous delivery can be administered in the leg, abdomen or arm. For intramuscular delivery, there are five main sites:
- Thigh (vastus lateralis, rectus femoris)
- Gluteal (ventrogluteal, dorsogluteal)
- Arm (deltoid).
The vastus lateralis (VL) is the largest of the four muscles forming the quadricep, located in the thigh. Its main function is to control the movement of the knee, working with surrounding tissue to stabilise the joint. The significant work done by the VL requires high levels of vascularisation; the femoral artery and other branching arteries, such as the profunda femoris, are key blood supplies. This makes the VL an ideal site for intramuscular injection, including for crisis medication. The thigh consists of different tissue structures, including:
- Skin (dermis and epidermis)
- Subcutaneous tissue
- Muscle, (vastus lateralis, rectus femoris, etc)
- Bone (femur).
For gluteal intramuscular injections, the ventrogluteal and dorsogluteal sites are shown in Figure 4. The selection is based on the formulation, dose volume, viscosity and patient considerations, such as thickness of subcutaneous fat layer.
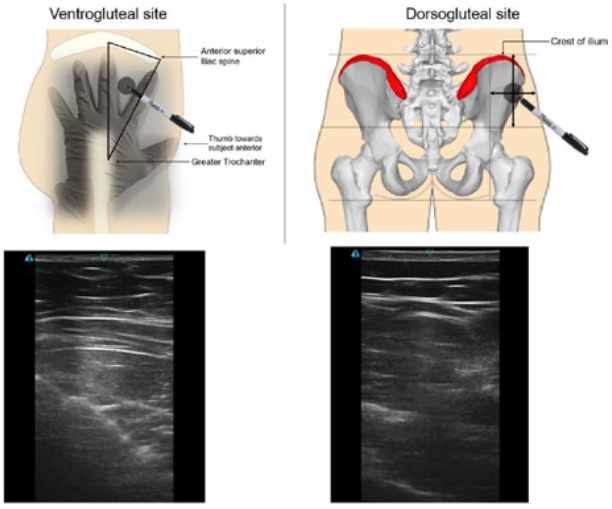
Figure 4: Identification of gluteal locations and sample ultrasound images.
IMPORTANCE OF NEEDLE LENGTH
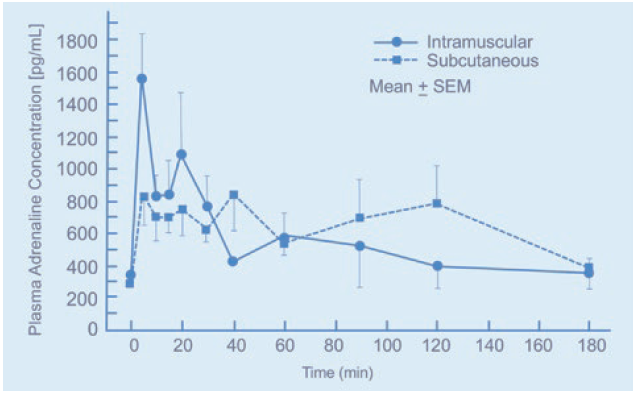
Figure 5: Plasma adrenaline concentration for intramuscular and subcutaneous injection over time.1
Consideration of the anatomical injection depth (subcutaneous or intramuscular) should be taken into account during the design of a drug delivery device to ensure optimal delivery of the drug, which is determined by the exposed needle length. Characterisation of the needle length requires an understanding of the STMD and STBD at the site of injection. Insufficient knowledge about tissue behaviour and compression can result in the drug being delivered into the incorrect tissue, which can have devastating consequences for the patient.
In crisis applications, autoinjectors are used for delivering adrenaline during anaphylactic shock. The pharmacokinetic (PK) properties of adrenaline require a high blood plasma concentration (Cmax); therefore, intramuscular administration is recommended into the VL. If adrenaline is delivered for the subcutaneous tissue, there may be a lag in the Cmax (Figure 5). Again, this can have devastating consequences for the patient.
On the other hand, the lag in Cmax means that long-acting injectables, such as insulin, are best administered subcutaneously. If the drug is delivered into the muscle, there will be a spike in blood plasma concentration; for insulin, this can cause the patient to become hypoglycaemic and reduce the length of time the drug is effective.
In the case of intramuscularly administered long-acting injectables, controlled release of the API is managed by adjusting solubility in a carrier. Due to the extended periods between administrations, it is essential that the drug is delivered to the correct depth in the muscle tissue, or it can result in the failure of the therapy.
“Similar to the FEA results, the ultrasound data showed that the muscle is responsible for the majority of tissue compression when the load is applied.”
POPULATION DISCREPANCIES
The distribution of subcutaneous tissue varies between males and females, with men commonly carrying it around their abdomen and women around their buttock and thighs. When considering an intramuscular injection into the VL, the chances of women receiving an erroneous subcutaneous injection are increased. Likewise, for a male targeting a subcutaneous injection into the quadriceps, a lack of subcutaneous fat may lead to an intramuscular injection. Therefore, variations in tissue thickness must be considered when designing an FEA simulation study. Other factors, such as weight and age, are characteristics that can lead to dissimilarities between patients.
TISSUE MECHANICS
Human tissues are highly complex, with interesting mechanical properties and structures; it is important to understand the architecture and physical characteristics of the tissue in question to ensure the accuracy of an FEA model. Human tissue is a hyper-viscoelastic material, meaning that deformation is highly non-linear, significant and time-dependent. Within the context of an FEA simulation, the tissue should be considered as solely a hyperelastic material, because the rate at which the load is applied remains constant.
Many variables can affect the structure and mechanical properties of human tissue, including age, sex and injury. For simplicity, the current model does not consider the difference these properties may have on the tissue, nor does it consider natural variation between subjects. Although, where possible, material properties were found for males and females individually.
TISSUE CHARACTERISATION WITH ULTRASOUND
A clinical study used ultrasound and a load cell to collect images of the anterolateral thigh and plot a force-time graph during compression of the tissue (Figure 6). Data collected from the ultrasound study assisted in developing the model and material characteristics. STMD, STBD and tissue deformation data during application of the probe were acquired using live imaging and load cell monitoring.
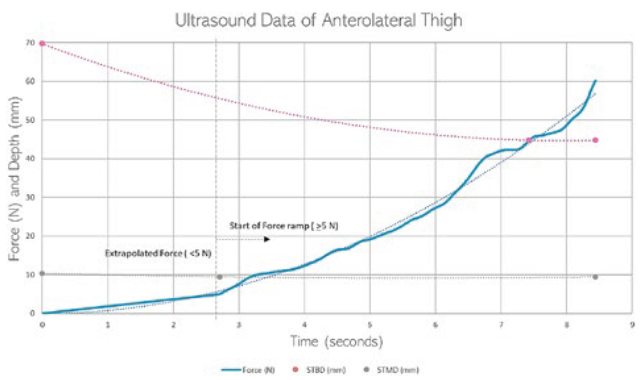
Figure 6: Graph showing an anterolateral thigh ultrasound measurement.
FINITE ELEMENT ANALYSIS
ABAQUS CAE 2021 (ABAQUS, CA, US) was used to create six models of human tissue, each representing a different patient group – both male and female for small, medium and large participants, categorised by BMI. This ensured that the data collected accounted for differences between sexes and the effect of tissue thickness on tissue compression. The surface area of the external load in the model matched the ultrasound probe used to gather the ultrasound data. The leg was modelled as a cylinder and then partitioned according to the tissue thickness calculated from the ultrasound study. Partitioning of the model into the individual tissue layers allows for the different material properties to be applied to each partition.
For the FEA simulation study, materials were considered to be homogenous and isotropic. Initially, linear elastic material properties were applied to the individual tissue layers to verify that the model works while keeping computation time to a minimum. Once verified, the model was tailored by applying hyperelastic material properties to the individual layers, allowing the model to consider the large deformations observed in ultrasound footage.
Various interactions were used to detail how the load simulating the ultrasound probe and human tissue behaved in relation to one another during the simulation. A standard contact-to-contact interaction was placed on the top surface of the human tissue and the outer surfaces of the probe. This allowed the tissue to deform when in contact with the probe. To simplify the model, only 1/8th of the leg was taken and symmetrical boundary conditions were applied to the model to represent the entire leg fully during calculation. The behaviour of bone was mimicked using a boundary condition not allowing for any displacement of the central partition. Finally, a displacement condition was implemented to represent axial translation of the probe onto the injection site.
The model used a varied hex mesh that decreased in size as it converged towards the probe location. This helped to balance computation time with accuracy – a finer mesh results in more calculations, therefore increasing the accuracy, but also results in a significantly longer computation time. Hence, it is advantageous to have a non-uniform mesh distribution across the model that becomes more accurate around the point of contact.
The results showed that each tissue behaves differently when a compressive force is applied; muscle accounts for the majority of tissue compression, with compression of the skin and subcutaneous tissue being minimal to non-existent. Figure 7 shows the small female muscle compressed by 13.75 mm, subcutaneous tissue by 1.25 mm and skin by 0 mm. It can also be seen that the high compression and Poisson’s ratio caused significant perpendicular movement of the tissue.
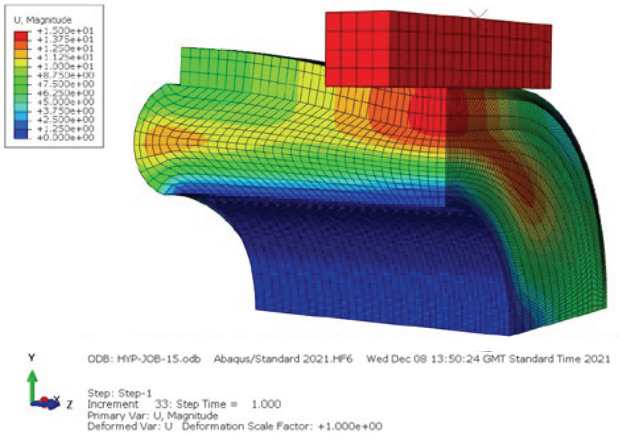
Figure 7: FEA results of a small female showing displacement in the simulation.
CLINICAL ULTRASOUND VERIFICATION
The ultrasound video footage was sampled to acquire tissue compression over time during application of the ultrasound probe. The load cell data was sampled to acquire force data over time during application, and the two were aligned with respect to one another to assess tissue compression at different forces. These data were used to verify the FEA model, ensuring that the model is representative of how real tissue behaves.
Similar to the FEA results, the ultrasound data showed that the muscle is responsible for the majority of tissue compression when the load is applied. It also showed that the muscle reaches a point of maximum compression, after which increasing force will not affect tissue compression. These data were used to guide the compression depth in the FEA model.
The force-displacement graph of the ultrasound mirrors that of the FEA, with only slight variability between the exact data values. To improve the similarity between the FEA data and ultrasound data, the tissue material properties will be iteratively changed to become more accurate. The change in material properties is intended to absorb the effect that surrounding tissues have on compression, for example, the tension of muscle or blood pressure.
CONCLUSION
The FEA simulation has shown the potential of FEA to create accurate models of human tissue, although further development of the model would be required before the results can be used as part of an autoinjector design. Unlike ultrasound, FEA can be used to understand the effect of skin doming on tissue thickness for different needle hole diameters. It can also be used to help improve the understanding of what data and participants are required, in terms of sex and size, to gather the necessary data for clinical trial design.
Using data from such studies, Oval has optimised its intramuscular and subcutaneous platforms to align the activation force with known tissue compression, thereby optimising needle length for various injection sites, population groups and challenging applications. The correlation between delivery performance and needle depth consistency over various time points and conditioning scenarios has been proven in technology and PK studies. Ensuring the correct needle length leads to more successful injections, creating a more patient-centric autoinjector.
The potential to use FEA to simulate novel and interesting situations opens exciting research and design opportunities that can improve on the current knowledge base surrounding the use of autoinjectors and, ultimately, the usability of devices.
REFERENCE
- Simons FE et al, “Epinephrine Absorption in Children with a History of Anaphylaxis”. J Allergy Clin Immunol, Jan 1998, Vol 101(1 Pt 1), pp 33–37.